Retroviruses undergo an obligatory maturation step in the formation of an infectious particle. Core particles either assembled in the cytoplasm or at the plasma membrane have an immature morphology. Except in the case of spumaviruses, which show little processing and retain an immature morphology, a condensed core is formed upon leaving the cell. Condensation is associated with the formation of an infectious viral particle. Processing of the Gag and Gag-Pro-Pol precursors by the viral PR is intimately involved in maturation of the viral particle (for a recent review, see Vogt 1996).
Coincidence of and Requirement for Processing and Morphologic Changes
There is an absolute requirement for protein processing in the formation of infectious virus. Virus generated from a genome with a mutation in the PR-coding domain (Crawford and Goff 1985; Katoh et al. 1985; Kohl et al. 1988; Stewart et al. 1990; Sommerfelt et al. 1992) or virus produced in the presence of a virus-specific PR inhibitor (Ashorn et al. 1990; Erickson et al. 1990; Meek et al. 1990; Roberts et al. 1990; Sommerfelt et al. 1992) contains unprocessed Gag and Gag-Pro-Pol proteins and is not infectious. The most visually striking feature of processing is the resulting change in virion structure. Virions formed in the absence of processing have an immature morphology most likely due to the continued anchoring of the Gag precursor to the inner face of the viral envelope through MA (Bolognesi et al. 1978; Gelderblom et al. 1987; Goto et al. 1990; Menendez-Arias et al. 1992). This is in contrast to virions formed with processed proteins where an electrondense core is largely detached from the membrane. This difference in morphology between virions containing processed and unprocessed proteins has been observed both for viruses that assemble at the plasma membrane (e.g., MLV: Luftig and Yoshinaka 1978; Katoh et al. 1985; HIV-1: Göttlinger et al. 1989; Peng et al. 1989; ASLV: Voynow and Coffin 1985; Stewart et al. 1990) and for particles that preassemble in the cytoplasm and then bud through the membrane (e.g., M-PMV: Sommerfelt et al. 1992) (see Figs. 4 and 15).
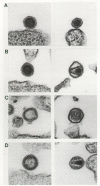
Figure 15
Changes in virion morphology with proteolytic processing. Immature and mature forms of viral particles are shown in pairs. (A) MLV; (B) M-PMV; (C) HTLV-1; (D) HIV-2. (Courtesy of M. Gonda and reprinted, with permission, from Coffin 1992.)
The immature cores produced in the absence of processing in ASLV (Stewart et al. 1990), MLV (Yoshinaka and Luftig 1977b), and HIV-1 (Park and Morrow 1993; Kaplan et al. 1994b) systems are physically different from their processed counterparts in that the core of the particle, after removal of the membrane envelope, is more stable to nonionic detergent in the unprocessed state. Converting more stable cores to less stable cores may represent a necessary transition from the requirements of assembly to the requirements of uncoating during the subsequent round of infection. For HIV-1, it has been shown that processing must be nearly complete for virions to be infectious and have normal morphology (Kaplan et al. 1993; Kageyama et al. 1994). Similarly, HIV-1 mutants with a mutant PR that expresses 20–25% wild-type activity are able to form infectious virus (Konvalinka et al. 1995c; Rose et al. 1995), whereas those with only 2% of the wild-type PR activity are not (Rose et al. 1995). Conversely, an overactive PR placed in ASLV results in the assembly of a core with a more diffuse appearance (Sedlacek et al. 1993).
Virions at the latest stages of budding usually have a distended, immature morphology (see Fig. 4). Given that this is the same morphology as a budded virion containing unprocessed protein, it would seem that processing must be delayed until after budding. However, in numerous systems, processing of the Gag precursor is clearly initiated in the cell, as evidenced by processed viral proteins associated with the cell (for review, see Dickson et al. 1984; see also Vogt et al. 1975; Kaplan and Swanstrom 1991). This observation suggests that processing may be initiated within the cell but that the morphological changes in the core do not occur until the time of virus release or shortly thereafter.
Several lines of evidence suggest that the cleavage of Gag that is detected within the cell occurs in the context of viral budding. First, most of the proteolytic cleavages of Gag appear to occur either before release from the cell or very rapidly after release, since viral particles are composed mostly of the mature products of Gag from the earliest times after release from the cell (Kaplan et al. 1994a). This is not true of all Gag processing events since some do occur more slowly and can be detected in budded viral particles (see, e.g., Eisenman et al. 1980; Pepinsky et al. 1986; Craven et al. 1993). Second, a PR inhibitor can slow the release of HIV-1 particles from the cell (Kaplan et al. 1994a), suggesting that under some circumstances, efficient budding is enhanced by proteolytic processing, although this is not the case with ASLV (J. Wills, unpubl.). Third, an interplay between budding and PR activity is suggested by the observations that inhibition of the PR reverses the reduced budding phenotype of an HIV-1 Gag p6 mutant (Huang et al. 1995) and that accelerated cleavage at a slowly cleaved site in ASLV Gag can, under some circumstances, reduce budding (Xiang et al. 1997).
Efforts to use electron microscopy to identify intermediates in the maturation of HIV-1 have yielded conflicting results, with evidence either for maturation to form the condensed core after budding (Palmer et al. 1985; Gelderblom et al. 1989; Katsumoto et al. 1990; Höglund et al. 1992) or for maturation during the late stages of budding (Goto et al. 1990). Post-budding maturation has also been reported for avian myeloblastosis virus (AMV) (Korb et al. 1976).
Taken together, these results suggest that processing can initiate within the cell, yet the premature core structure is maintained until after budding, although this point is still debated. One can imagine a complex series of events involving changes in protein-protein interactions that result in efficient budding and a change in the organization of the processed core proteins. Most of the details of this dynamic process are still to be determined.
Ordered Processing
Ordered processing of Gag and Gag-Pro-Pol appears to be a general feature among different retroviruses (for review, see Dickson et al. 1984). This conclusion is based on experiments identifying steady-state intermediates and precursor-product relationships using pulse-chase strategies in infected cells and from cleavage of Gag in vitro. Despite many differences in detail, a comparison of ordered processing within Gag among diverse retroviruses, including ASLV (Eisenman et al. 1980; Burstein et al. 1992), MLV (Ledbetter 1979; Naso et al. 1979; Maxwell and Arlinghaus 1981; Yoshinaka and Luftig 1982), MMTV (Dickson and Atterwill 1978, 1979; Racevskis and Sarkar 1978; Massey and Schochetman 1979; Hizi et al. 1989), HTLV-1 (Hatanaka and Nam 1989), and HIV-1 (Kräusslich et al. 1988; Mervis et al. 1988; Erickson-Viitenan et al. 1989; Gowda et al. 1989), reveals several common features. Most notably, cleavage at the amino terminus of CA precedes cleavage at the carboxyl terminus of CA. At present, the significance of this apparently conserved ordered cleavage is not known.
Three levels of control have been identified thus far that regulate the rate of cleavage at the different sites. First is the sequence of the processing site itself; different cleavage site sequences placed in the same context have different rates of cleavage (Tritch et al. 1991; Pettit et al. 1994), and peptides representing various cleavage sites are cleaved at different rates (Billich et al. 1988; Darke et al. 1988; Kräusslich et al. 1989; Tozser et al. 1991; Schock et al. 1996). Second, the efficiency of cleavage at a site can change between the intact Gag precursor and the processing intermediates, indicating a role for context (Tritch et al. 1991; Pettit et al. 1994). Third, cleavage at specific sites can be accelerated by the presence of RNA, suggesting changes in rates of cleavage as RNA is packaged (Jamjoom et al. 1976; Sheng and Erickson-Viitenan 1994; Sheng et al. 1997).
Is the ordered cleavage of Gag important for proper maturation? At present, there is no direct answer to this question. Blocking cleavage at the MA-CA, CA-p2, or p2-NC site of HIV-1 is disruptive to replication and proper maturation (Göttlinger et al. 1989; Pettit et al. 1994; Kräusslich et al. 1995). Similarly, incomplete cleavage results in the formation of noninfectious virus with aberrant core structures (Kaplan et al. 1993; Kageyama et al. 1994). Thus, although the need for specific cleavages has been demonstrated along with the need for near complete cleavage, the role of ordered cleavage has yet to be defined.
Protease-mediated Processing of Env
As noted earlier (see above Synthesis and Organization of Env Glycoproteins; Transport, Cleavage, and Further Modifications of Env), a cellular protease in the Golgi apparatus cleaves the Env polyprotein to separate the SU sequence from TM, an event that is prerequisite for activation of the hydrophobic fusion peptide at the amino terminus of TM. This peptide sequence is needed after the mature virus is released and bound (via SU) to the next host cell. It is thought that the binding of SU may trigger a large conformational change in the structure of TM that results in the fusion peptide being buried into the opposing membrane, thereby allowing viral and cellular membranes to be pulled closer together to promote fusion (Chapter 3.
Because the fusion peptide is liberated well before the Env proteins arrive at the cell surface for packaging—at a point where they are already competent for binding to the receptor—the potential would seem to exist for nascent glycoproteins to mediate fusion between the virus-producing cells and adjacent uninfected cells (i.e., to induce syncytium formation). Indeed, cell-to-cell fusion is readily observed for some, but certainly not all, retroviruses (e.g., for HIV-1 but not ASLV). This suggests that some viruses possess mechanisms for regulating their fusion activity. In the model case of influenza virus (see, e.g., Bullough et al. 1994; Hughson 1995), fusion requires low pH conditions, a situation that is not encountered by the glycoproteins until after they are packaged into virions and invade the endocytic compartment of a newly infected cell. However, most retroviruses do not require low pH conditions for fusion (see, e.g., McClure et al. 1990), and therefore this explanation for an absence of syncytia cannot be applied to most retroviruses (Chapter 3.
Recently, a novel mechanism for regulating fusion has been identified for the Env proteins of two retroviruses, MLV and M-PMV. The glycoproteins of these viruses possess a PR cleavage site within the cytoplasmic domain of the TM sequence (see Fig. 9). This site is recognized not by a host protease but by the virus-encoded PR (Green et al. 1981; Henderson et al. 1984; Schultz and Rein 1985; Brody et al. 1992; Sommerfelt et al. 1992). Consequently, cleavage does not occur until after the Gag, Gag-Pro-Pol, and Env proteins come together in the budding particle and the viral PR is activated. Removal of the carboxy-terminal peptides by mutagenesis results in glycoproteins that are capable of inducing the formation of syncytia with adjacent uninfected cells (Brody et al. 1994b; Ragheb and Anderson 1994; Rein et al. 1994b). Thus, it appears that both cleavage of SU from TM and removal of the carboxy-terminal “R” peptide are necessary to activate the fusion activity of MLV and M-PMV glycoproteins. How the PR-mediated cleavage event exerts its effect from the opposite side of the viral membrane is not known. Moreover, the mechanisms by which other retroviral glycoproteins (e.g., those of ASLV) are prevented from inducing syncytia remain to be determined.
RNA Maturation
Viral RNA isolated from mature particles is in a dimeric form that is held together by weak forces, presumably including base pairing, that are maintained in the absence of viral proteins (see Chapter 2 and Fig. 16). The initial evidence on the state of viral RNA in newly budded virions of ASLV suggested that monomeric RNA is packaged and that this RNA matures in the budded virion to the dimeric form (Canaani et al. 1973; Korb et al. 1976). An alternative and more complex view was suggested by the observation that dimeric RNA could be detected in newly budded virions (Cheung et al. 1972) but that this RNA was in a less stable conformation relative to the older matured virions (Stoltzfus and Snyder 1975).
The issue of the presence of RNA dimers in newly budded virions has been reexamined (Fu and Rein 1993). Newly released MLV particles contain a dimeric form of viral RNA that dissociates to monomers at a lower temperature relative to dimeric RNA in older mature virions. These low-stability dimers also have reduced mobility during electrophoresis under nondenaturing conditions. The results indicate that viral RNA can exist in at least two distinct dimeric states and that there is a shift to a more stable dimer in the virion.
A role for Gag and Gag-Pro-Pol processing and for the NC protein in the maturation of viral RNA has been demonstrated. PR-deficient viruses assemble virions with dimeric RNA that is even less stable than that seen in newly budded virions (MLV: Fu and Rein 1993) or with RNA in a mixture of monomeric and dimeric forms (ASLV: Oertle and Spahr 1990; Stewart et al. 1990; HIV-1: Fu et al. 1994). The dimeric RNA that is formed is of an altered, low-mobility conformation, and the presence of monomeric RNA may represent dissociation of these low-stability dimer intermediates.
NC mutants have variable effects on RNA maturation. In one case, an MLV NC mutant that packages reduced levels of RNA was shown to have dimeric RNA in the low-mobility conformation (Fu and Rein 1993). Two ASLV NC mutants packaged dimeric RNA of slower electrophoretic mobility, and in one case, the packaged RNA was present in both monomeric and dimeric forms (Méric and Spahr 1986; Méric et al. 1988). Thus, both protein processing and an appropriate interaction with the NC protein are required for maturation of viral RNA to its mature dimeric form.
The primer tRNA appears to be placed on the RNA genome of ASLV subsequent to viral budding (Canaani et al. 1973), and annealing to the viral genome can take place in the absence of Gag and Gag-Pro-Pol precursor processing (Crawford and Goff 1985; Stewart et al. 1990; Kaplan et al. 1994b).
Attempts have been made to reproduce RNA maturation steps in vitro. These efforts have focused on formation of the dimer linkage structure (DLS) and accelerated strand association mediated by the NC protein. Initial observations of the structure of dimeric viral RNA by electron microscopy placed the most stable region of interaction between the two copies of viral RNA near the 5′end of the genome (Fig. 16) (Bender and Davidson 1976; Kung et al. 1976; Bender et al. 1978; Murti et al. 1981; Höglund et al. 1997). In several systems, it has been possible to show specific self-association of a region of viral RNA near the 5′end of the genome (MLV: Prats et al. 1990; Torrent et al. 1994; Feng et al. 1995; ASLV: Bieth et al. 1990; BLV: Katoh et al. 1991, 1993; REV: Darlix et al. 1992; and HIV-1: for examples, see Laughrea and Jette 1994; Skripkin et al. 1994; Clever et al. 1995 and references therein). Chemical probing has been used to show that dimerization of MLV RNA in vitro leads to changes in the reactivity of a subset of the bases, presumed to be a consequence of dimerization (Tounekti et al. 1992). Several reports have suggested that dimerization of HIV-1 RNA involves the formation of G quartets, four-stranded structures related to the structure of telomeric DNA (Awang and Sen 1993; Sundquist and Heaphy 1993). However, regions of HIV-1 and HIV-2 RNAs that lack the region forming G quartets can still form dimers in vitro (Darlix et al. 1990; Berkhout et al. 1993; Sakaguchi et al. 1993; Skripkin et al. 1994), and dimeric RNA isolated from HIV-1 virions does not appear to be stabilized by G quartets (Fu et al. 1994). A major determinant of HIV-1 RNA dimerization in vitro is a stem-loop structure (SL1), called the dimer initiation site or DIS, that is able to dimerize through a kissing loop mechanism (see Clever et al. 1996 and references therein). Mutations within this region have a significant effect on viral infectivity, a lesser effect on RNA packaging, and a variable effect on RNA dimerization within the virion (Berkhout and van Wamel 1996; McBride and Panganiban 1996; Paillart et al. 1996; Clever and Parslow 1997; Laughrea et al. 1997).
A possible role for viral proteins in accelerating both the dimerization of viral RNA and the placement of the primer tRNA on the genome has also been suggested from in vitro studies. The NC proteins from ASLV (Bieth et al. 1990), MLV (Prats et al. 1990), and HIV-1 (Darlix et al. 1990; De Rocquigny et al. 1992; Sakaguchi et al. 1993) have been shown to accelerate the dimerization of their homologous RNAs in vitro. With BLV, the p15 form of MA has a similar activity (Katoh et al. 1991). Similarly, NC can also accelerate the annealing of the tRNA primer to the genome (Prats et al. 1988), likely promoted by the ability of NC to unwind the tRNA structure (Khan and Giedroc 1992). Such an activity has also been suggested for RT (Araya et al. 1979). The RNA annealing activity (Prats et al. 1991; De Rocquigny et al. 1993) and tRNA unwinding activity (Khan and Giedroc 1992) of NC are not dependent on intact zinc finger structures but are mediated by basic residues found in NC.
The presence of viral RNA in the virion may contribute to normal morphogenesis. HIV-1 particles with altered core structures have been seen when the virus was generated from a genome that carried a deletion within the RNA packaging domain (Aldovini and Young 1990; Clavel and Orenstein 1990), although in an analogous study, normal virion structures were seen (Lever et al. 1989). An attractive model for the role of RNA in virion morphogenesis is that after release of NC from the Gag precursor, intra- and interstrand RNA annealing takes place, mediated by NC, until a highly compact core structure is attained.
Changes in Protein Activities after Gag and Gag-Pro-Pol Processing
Virtually all virion proteins function as dimers or higher-order oligomers; thus, the process of virion assembly is a necessary step in creating active proteins. The role of dimerization in forming the mature forms of RT and IN is discussed in Chapters 4 and 5, and the role of dimerization in forming active PR is discussed above. Beyond the assembly/oligomerization step, a number of proteins have been shown to have different activities in the precursor form compared to the processed form, implying that processing is required to generate the appropriately active form of these proteins. On a global level, the entire core structure made by unprocessed Gag and Gag-Pro-Pol precursors is much more stable compared to the core consisting of processed proteins (Yoshinaka and Luftig 1977b; Stewart et al. 1990; Park and Morrow 1993; Kaplan et al. 1994b). The HIV-1 CA protein likely undergoes significant structural changes after cleavage at the MA-CA cleavage site as inferred by the fact that the amino-terminal amino acid of CA Pro1 is involved in a buried salt bridge, rather than in the presumed exposed cleavage site that exists prior to cleavage (Gitti et al. 1996). Similarly, ASLV CA proteins have distinct interactions prior to and after proteolytic processing (Pepinsky et al. 1980; Pepinsky 1983). In addition, in ASLV virions assembled from unprocessed precursors, the NC protein makes different (either fewer or looser) contacts with viral RNA compared to the contacts made by mature NC with viral RNA (Stewart et al. 1990).
The HIV-1 protease is clearly active in the context of a precursor molecule, either when linked to the downstream Pol polyprotein (Debouck et al. 1987; Farmerie et al. 1987; Le Grice et al. 1987; Mous et al. 1988; Loeb et al. 1989a) or when linked to the upstream Gag sequences (Kräusslich et al. 1988). However, processing of the precursor also releases free PR and this may lead to overestimates of the activity of PR in the precursor. Mutations that block the amino-terminal processing site of the HIV-1 PR linking it to the upstream pro reading frame sequences (Loeb et al. 1989a; Kotler et al. 1992; Phylip et al. 1992) or to part or all of the upstream Gag sequences (Kotler et al. 1992; Zybarth et al. 1994) give an active PR in vitro, but such a mutation in either HIV-1 or ASLV renders the virus noninfectious (Oertle and Spahr 1990; Burstein et al. 1992; Zybarth et al. 1994). Surprisingly, a p6-PR intermediate is readily detected in HIV-1 particles (Almog et al. 1996). Mutations that block cleavage at the downstream PR/RT cleavage site severely limit cleavage at downstream cleavage sites in Pol (Loeb et al. 1989a). HIV-1 PR extended at either its amino or carboxyl terminus has reduced activity on polyprotein substrates in vitro (Louis et al. 1991; Zybarth et al. 1994). Thus, although the protease has some activity in the precursor form, it is clear that it must be released from the precursor to be fully effective in processing the Gag and Gag-Pro-Pol precursors.
RT from several retroviruses has also been reported to be active in the precursor form when assayed in disrupted virions composed of unprocessed proteins. However, the amount of residual RT activity observed for the Gag-Pro-Pol precursor varies in different reports and for different retroviruses, from near wild type to very low levels (Witte and Baltimore 1978; Crawford and Goff 1985; Panet and Baltimore 1987; Stewart et al. 1990; Craven et al. 1991; Peng et al. 1991; Schätzl et al. 1991; Kaplan et al. 1994b). Particles composed of unprocessed proteins fail to carry out DNA synthesis when used to infect permissive cells, suggesting a block in infection at an early step (Crawford and Goff 1985; Kaplan et al. 1994b). RT embedded in the Gag-Pro-Pol precursor or in processing intermediates can also be active as purified protein. However, the amount of activity is very dependent on the constellation of flanking protein domains (Lori et al. 1988; Hu and Kang 1991; Stewart and Vogt 1993). A comparison of IN activity in the Gag-Pro-Pol precursor has not been reported. However, in the case of ASLV, IN is active as part of the β-subunit of RT (Duyk et al. 1983), and an incompletely processed form of IN with a 40-amino-acid carboxy-terminal extension is also active (Terry et al. 1988).
Publication Details
Copyright
Publisher
Cold Spring Harbor Laboratory Press, Cold Spring Harbor (NY)
NLM Citation
Coffin JM, Hughes SH, Varmus HE, editors. Retroviruses. Cold Spring Harbor (NY): Cold Spring Harbor Laboratory Press; 1997. Maturation of Viral Particles.